The world of optical communication is undergoing a transformation with the introduction of Hollow Core Fiber (HCF) technology. This revolutionary technology offers an alternative to traditional Single Mode Fiber (SMF) and presents exciting new possibilities for improving data transmission, reducing costs, and enhancing overall performance. In this article, we will explore the benefits, challenges, and applications of HCF, providing a clear and concise guide for optical fiber engineers.
What is Hollow Core Fiber (HCF)?
Hollow Core Fiber (HCF) is a type of optical fiber where the core, typically made of air or gas, allows light to pass through with minimal interference from the fiber material. This is different from Single Mode Fiber (SMF), where the core is made of solid silica, which can introduce problems like signal loss, dispersion, and nonlinearities.
In HCF, light travels through the hollow core rather than being confined within a solid medium. This design offers several key advantages that make it an exciting alternative for modern communication networks.
Traditional SMF vs. Hollow Core Fiber (HCF)
Single Mode Fiber (SMF) technology has dominated optical communication for decades. Its core is made of silica, which confines laser light, but this comes at a cost in terms of:
- Attenuation: SMF exhibits more than 0.15 dB/km attenuation, necessitating Erbium-Doped Fiber Amplifiers (EDFA) or Raman amplifiers to extend transmission distances. However, these amplifiers add Amplified Spontaneous Emission (ASE) noise, degrading the Optical Signal-to-Noise Ratio (OSNR) and increasing both cost and power consumption.
- Dispersion: SMF suffers from chromatic dispersion (CD), requiring expensive Dispersion Compensation Fibers (DCF) or power-hungry Digital Signal Processing (DSP) for compensation. This increases the size of the transceiver (XCVR) and overall system costs.
- Nonlinearity: SMFâs inherent nonlinearities limit transmission power and distance, which affects overall capacity. Compensation for these nonlinearities, usually handled at the DSP level, increases the systemâs complexity and power consumption.
- Stimulated Raman Scattering (SRS): This restricts wideband transmission and requires compensation mechanisms at the amplifier level, further increasing cost and system complexity.
In contrast, Hollow Core Fiber (HCF) offers significant advantages:
- Attenuation: Advanced HCF types, such as Nested Anti-Resonant Nodeless Fiber (NANF), achieve attenuation rates below 0.1 dB/km, especially in the O-band, matching the performance of the best SMF in the C-band.
- Low Dispersion and Nonlinearity: HCF exhibits almost zero CD and nonlinearity, which eliminates the need for complex DSP systems and increases the systemâs capacity for higher-order modulation schemes over long distances.
- Latency: The hollow core reduces latency by approximately 33%, making it highly attractive for latency-sensitive applications like high-frequency trading and satellite communications.
- Wideband Transmission: With minimal SRS, HCF allows ultra-wideband transmission across O, E, S, C, L, and U bands, making it ideal for next-generation optical systems.
Operational Challenges in Deploying HCF
Despite its impressive benefits, HCF also presents some challenges that engineers need to address when deploying this technology.
1. Splicing and Connector Challenges
Special care must be taken when connecting HCF cables. The hollow core can allow air to enter during splicing or through connectors, which increases signal loss and introduces nonlinear effects. Special connectors are required to prevent air ingress, and splicing between HCF and SMF needs careful alignment to avoid high losses. Fortunately, methods like thermally expanded core (TEC) technology have been developed to improve the efficiency of these connections.
2. Amplification Issues
Amplifying signals in HCF systems can be challenging due to air-glass reflections at the interfaces between different fiber types. Special isolators and mode field couplers are needed to ensure smooth amplification without signal loss.
3. Bend Sensitivity
HCF fibers are more sensitive to bending than traditional SMF. While this issue is being addressed with new designs, such as Photonic Crystal Fibers (PCF), engineers still need to handle HCF with care during installation.
4. Fault Management
HCF has a lower back reflection compared to SMF, which makes it harder to detect faults using traditional Optical Time Domain Reflectometry (OTDR). New low-cost OTDR systems are being developed to overcome this issue, offering better fault detection in HCF systems.
(a) Schematics of a 3Ă4-slot mating sleeve and two CTF connectors; (b) principle of lateral offset reduction by using a multi-slot mating sleeve; (c) Measured ILs (at 1550 nm) of a CTF/CTF interconnection versus the relative rotation angle; (d) Minimum ILs of 10 plugging trials.
Applications of Hollow Core Fiber
HCF is already being used in several high-demand applications, and its potential continues to grow.
1. Financial Trading Networks
HCFâs low-latency properties make it ideal for high-frequency trading (HFT) systems, where reducing transmission delay can provide a competitive edge. The London Stock Exchange has implemented HCF to speed up transactions, and this use case is expanding across financial hubs globally.
2. Data Centers
The increasing demand for fast, high-capacity data transfer in data centers makes HCF an attractive solution. Anti-resonant HCF designs are being tested for 800G applications, which significantly reduce the need for frequent signal amplification, lowering both cost and energy consumption.
3. Submarine Communication Systems
Submarine cables, which carry the majority of international internet traffic, benefit from HCFâs low attenuation and high power transmission capabilities. HCF can transmit kilowatt-level power over long distances, making it more efficient than traditional fiber in submarine communication networks.
4. 5G Networks and Remote Radio Access
As 5G networks expand, Remote Radio Units (RRUs) are increasingly connected to central offices through HCF. HCFâs ability to cover larger geographic areas with low latency helps 5G providers increase their coverage while reducing costs. This technology also allows networks to remain resilient, even during outages, by quickly switching between units.
Future Directions for HCF Technology
HCF is poised to shift the focus of optical transmission from the C-band to the O-band, thanks to its ability to maintain low chromatic dispersion and attenuation in this frequency range. This shift could reduce costs for long-distance communication by simplifying the required amplification and signal processing systems.
In addition, research into high-power transmission through HCF is opening up new opportunities for applications that require the delivery of kilowatts of power over several kilometers. This is especially important for data centers and other critical infrastructures that need reliable power transmission to operate smoothly during grid failures.
Hollow Core Fiber (HCF) represents a leap forward in optical communication technology. With its ability to reduce latency, minimize signal loss, and support high-capacity transmission over long distances, HCF is set to revolutionize industries from financial trading to data centers and submarine networks.
While challenges such as splicing, amplification, and bend sensitivity remain, the ongoing development of new tools and techniques is making HCF more accessible and affordable. For optical fiber engineers, understanding and mastering this technology will be key to designing the next generation of communication networks.
As HCF technology continues to advance, it offers exciting potential for building faster, more efficient, and more reliable optical networks that meet the growing demands of our connected world.
References/Credit :
- Image https://www.holightoptic.com/what-is-hollow-core-fiber-hcf%EF%BC%9F/Â
- https://www.mdpi.com/2076-3417/13/19/10699
- https://opg.optica.org/oe/fulltext.cfm?uri=oe-30-9-15149&id=471571
- https://www.ofsoptics.com/a-hollow-core-fiber-cable-for-low-latency-transmission-when-microseconds-count/
In this ever-evolving landscape of optical networking, the development of coherent optical standards, such as 400G ZR and ZR+, represents a significant leap forward in addressing the insatiable demand for bandwidth, efficiency, and scalability in data centers and network infrastructure. This technical blog delves into the nuances of these standards, comparing their features, applications, and how they are shaping the future of high-capacity networking. ZR stands for “Ze Best Range” and ZR+ is reach “Ze Best Range plus”
Introduction to 400G ZR
The 400G ZR standard, defined by the Optical Internetworking Forum (OIF), is a pivotal development in the realm of optical networking, setting the stage for the next generation of data transmission over optical fiber’s. It is designed to facilitate the transfer of 400 Gigabit Ethernet over single-mode fiber across distances of up to 120 kilometers without the need for signal amplification or regeneration. This is achieved through the use of advanced modulation techniques like DP-16QAM and state-of-the-art forward error correction (FEC).
Key features of 400G ZR include:
- High Capacity:Â Supports the transmission of 400 Gbps using a single wavelength.
- Compact Form-Factor:Â Integrates into QSFP-DD and OSFP modules, aligning with industry standards for data center equipment.
- Cost Efficiency:Â Reduces the need for external transponders and simplifies network architecture, lowering both CAPEX and OPEX.
Emergence of 400G ZR+
Building upon the foundation set by 400G ZR, the 400G ZR+ standard extends the capabilities of its predecessor by increasing the transmission reach and introducing flexibility in modulation schemes to cater to a broader range of network topologies and distances. The OpenZR+ MSA has been instrumental in this expansion, promoting interoperability and open standards in coherent optics.
Key enhancements in 400G ZR+ include:
- Extended Reach:Â With advanced FEC and modulation, ZR+ can support links up to 2,000 km, making it suitable for longer metro, regional, and even long-haul deployments.
- Versatile Modulation:Â Offers multiple configuration options (e.g., DP-16QAM, DP-8QAM, DP-QPSK), enabling operators to balance speed, reach, and optical performance.
- Improved Power Efficiency:Â Despite its extended capabilities, ZR+ maintains a focus on energy efficiency, crucial for reducing the environmental impact of expanding network infrastructures.
ZR vs. ZR+: A Comparative Analysis
Feature. | 400G ZR | 400G ZR+ |
---|---|---|
Reach | Up to 120 km | Up to 2,000 km |
Modulation | DP-16QAM | DP-16QAM, DP-8QAM, DP-QPSK |
Form Factor | QSFP-DD, OSFP | QSFP-DD, OSFP |
Application | Data center interconnects | Metro, regional, long-haul |
Adding few more interesting table for readers
Based on application
Product | Reach | Client Formats | Data Rate & Modulation | Wavelength | Tx Power | Connector | Fiber | Interoperability | Application |
800G ZR+ | 4000 km+ | 100GbE 200GbE 400GbE 800GbE |
800G Interop PCSÂ Â 600G PCSÂ Â 400G PCS |
1528.58 to  1567.34 |
>+1 dBm (with TOF) | LC | SMF | OpenROADM interoperable PCS | Ideal for metro/regional Ethernet data center and service provider network interconnects |
800ZR | 120 km | 100GbE 200GbE 400GbE |
800G 16QAMÂ Â 600G PCSÂ Â 400G Interop QPSK/16QAMÂ Â PCS |
1528.58 to  1567.34 |
-11 dBm to -2 dBm | LC | SMF | OIF 800ZR Â OpenROADM Interop PCS Â OpenZR+ |
Ideal for amplified single-span data center interconnect applications |
400G Ultra Long Haul | 4000 km+ | 100GbE 200GbE 400GbE |
400G Interoperable QPSK/16QAMÂ Â PCS |
1528.58 to  1567.34 |
>+1 dBm (with TOF) | LC | SMF | OpenROADM Interop PCS | Ideal for long haul and ultra-long haul service provider ROADM network applications |
Bright 400ZR+ | 4000 km+ | 100GbE 200GbE 400GbE OTUCn OTU4 |
400G 16QAMÂ Â 300G 8QAMÂ Â 200G/100G QPSK |
1528.58 to  1567.34 |
>+1 dBm (with TOF) | LC | SMF | OpenZR+ Â OpenROADM |
Ideal for metro/regional and service provider ROADM network applications |
400ZR | 120 km | 100GbE 200GbE 400GbE |
400G 16QAM | 1528.58 to  1567.34 |
>-10 dBm | LC | SMF | OIF 400ZR | Ideal for amplified single span data center interconnect applications |
OpenZR+ | 4000 km+ | 100GbE 200GbE 400GbE |
400G 16QAMÂ Â 300G 8QAMÂ Â 200G/100G QPSK |
1528.58 to  1567.34 |
>-10 dBm | LC | SMF | OpenZR+ Â OpenROADM |
Ideal for metro/regional Ethernet data center and service provider network interconnects |
400G ER1 | 45 km | 100GbE 400GbE |
400G 16QAM | Fixed C to band |
>12.5 dB Link Budget | LC | SMF | OIF 400ZR application code 0x02 Â OpenZR+ |
Ideal for unamplified point-to-point links |
*TOF: Tunable Optical Filter
The Future Outlook
The advent of 400G ZR and ZR+ is not just a technical upgrade; it’s a paradigm shift in how we approach optical networking. With these technologies, network operators can now deploy more flexible, efficient, and scalable networks, ready to meet the future demands of data transmission.
Moreover, the ongoing development and expected introduction of XR optics highlight the industry’s commitment to pushing the boundaries of what’s possible in optical networking. XR optics, with its promise of multipoint capabilities and aggregation of lower-speed interfaces, signifies the next frontier in coherent optical technology.
Reference
Acacia Introduces 800ZR and 800G ZR+ with Interoperable PCS in QSFP-DD and OSFP
Optical Amplifiers (OAs) are key parts of today’s communication world. They help send data under the sea, land and even in space .In fact it is used in all electronic and telecommunications industry which has allowed human being develop and use gadgets and machines in daily routine.Due to OAs only; we are able to transmit data over a distance of few 100s too 1000s of kilometers.
Classification of OA Devices
Optical Amplifiers, integral in managing signal strength in fiber optics, are categorized based on their technology and application. These categories, as defined in ITU-T G.661, include Power Amplifiers (PAs), Pre-amplifiers, Line Amplifiers, OA Transmitter Subsystems (OATs), OA Receiver Subsystems (OARs), and Distributed Amplifiers.
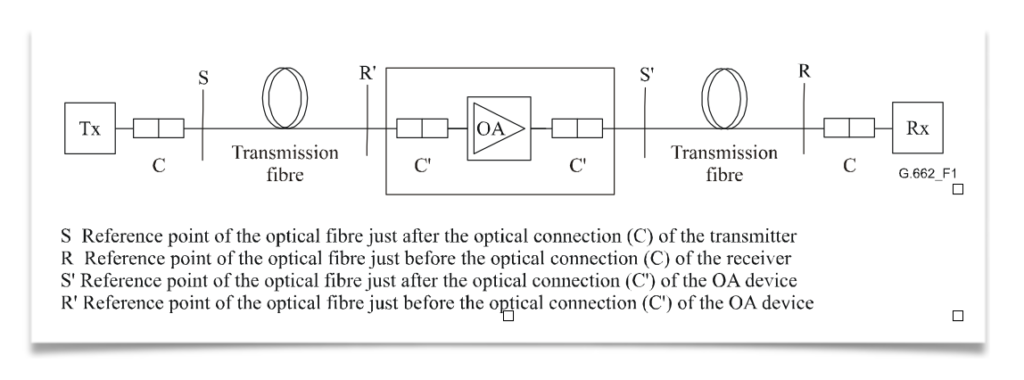
Scheme of insertion of an OA device
- Power Amplifiers (PAs): Positioned after the optical transmitter, PAs boost the signal power level. They are known for their high saturation power, making them ideal for strengthening outgoing signals.
- Pre-amplifiers: These are used before an optical receiver to enhance its sensitivity. Characterized by very low noise, they are crucial in improving signal reception.
- Line Amplifiers: Placed between passive fiber sections, Line Amplifiers are low noise OAs that extend the distance covered before signal regeneration is needed. They are particularly useful in point-multipoint connections in optical access networks.
- OA Transmitter Subsystems (OATs): An OAT integrates a power amplifier with an optical transmitter, resulting in a higher power transmitter.
- OA Receiver Subsystems (OARs): In OARs, a pre-amplifier is combined with an optical receiver, enhancing the receiver’s sensitivity.
- Distributed Amplifiers: These amplifiers, such as those using Raman pumping, provide amplification over an extended length of the optical fiber, distributing amplification across the transmission span.
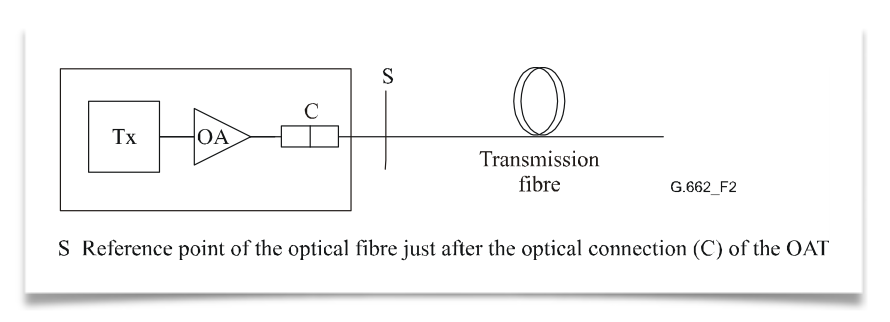
Scheme of insertion of an OAT
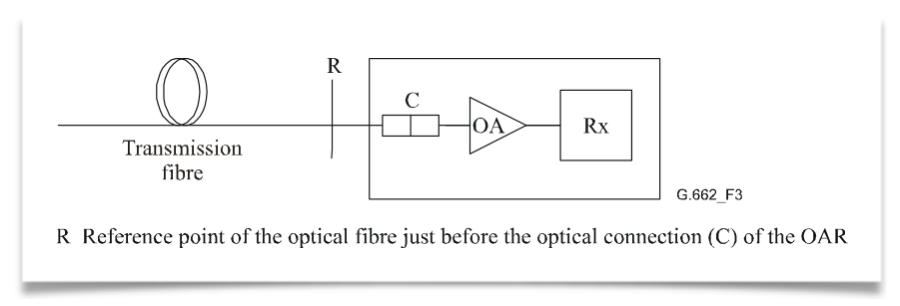
Applications and Configurations
The application of these OA devices can vary. For instance, a Power Amplifier (PA) might include an optical filter to minimize noise or separate signals in multiwavelength applications. The configurations can range from simple setups like Tx + PA + Rx to more complex arrangements like Tx + BA + LA + PA + Rx, as illustrated in the various schematics provided in the IEC standards.
Building upon the foundational knowledge of Optical Amplifiers (OAs), it’s essential to understand the practical configurations of these devices in optical networks. According to the definitions of Booster Amplifiers (BAs), Pre-amplifiers (PAs), and Line Amplifiers (LAs), and referencing Figure 1 from the IEC standards, we can explore various OA device applications and their configurations. These setups illustrate how OAs are integrated into optical communication systems, each serving a unique purpose in enhancing signal integrity and network performance.
- Tx + BA + Rx Configuration: This setup involves a transmitter (Tx), followed by a Booster Amplifier (BA), and then a receiver (Rx). The BA is used right after the transmitter to increase the signal power before it enters the long stretch of the fiber. This configuration is particularly useful in long-haul communication systems where maintaining a strong signal over vast distances is crucial.
- Tx + PA + Rx Configuration: Here, the system comprises a transmitter, followed by a Pre-amplifier (PA), and then a receiver. The PA is positioned close to the receiver to improve its sensitivity and to amplify the weakened incoming signal. This setup is ideal for scenarios where the incoming signal strength is low, and enhanced detection is required.
- Tx + LA + Rx Configuration: In this configuration, a Line Amplifier (LA) is placed between the transmitter and receiver. The LA’s role is to amplify the signal partway through the transmission path, effectively extending the reach of the communication link. This setup is common in both long-haul and regional networks.
- Tx + BA + PA + Rx Configuration: This more complex setup involves both a BA and a PA, with the BA placed after the transmitter and the PA before the receiver. This combination allows for both an initial boost in signal strength and a final amplification to enhance receiver sensitivity, making it suitable for extremely long-distance transmissions or when signals pass through multiple network segments.
- Tx + BA + LA + Rx Configuration: Combining a BA and an LA provides a powerful solution for extended reach. The BA boosts the signal post-transmission, and the LA offers additional amplification along the transmission path. This configuration is particularly effective in long-haul networks with significant attenuation.
- Tx + LA + PA + Rx Configuration: Here, the LA is used for mid-path amplification, while the PA is employed near the receiver. This setup ensures that the signal is sufficiently amplified both during transmission and before reception, which is vital in networks with long spans and higher signal loss.
- Tx + BA + LA + PA + Rx Configuration: This comprehensive setup includes a BA, an LA, and a PA, offering a robust solution for maintaining signal integrity across very long distances and complex network architectures. The BA boosts the initial signal strength, the LA provides necessary mid-path amplification, and the PA ensures that the receiver can effectively detect the signal.
Characteristics of Optical Amplifiers
Each type of OA has specific characteristics that define its performance in different applications, whether single-channel or multichannel. These characteristics include input and output power ranges, wavelength bands, noise figures, reflectance, and maximum tolerable reflectance at input and output, among others.
For instance, in single-channel applications, a Power Amplifier’s characteristics would include an input power range, output power range, power wavelength band, and signal-spontaneous noise figure. In contrast, for multichannel applications, additional parameters like channel allocation, channel input and output power ranges, and channel signal-spontaneous noise figure become relevant.
Optically Amplified Transmitters and Receivers
In the realm of OA subsystems like OATs and OARs, the focus shifts to parameters like bit rate, application code, operating signal wavelength range, and output power range for transmitters, and sensitivity, overload, and bit error ratio for receivers. These parameters are critical in defining the performance and suitability of these subsystems for specific applications.
Understanding Through Practical Examples
To illustrate, consider a scenario in a long-distance fiber optic communication system. Here, a Line Amplifier might be employed to extend the transmission distance. This amplifier would need to have a low noise figure to minimize signal degradation and a high saturation output power to ensure the signal remains strong over long distances. The specific values for these parameters would depend on the system’s requirements, such as the total transmission distance and the number of channels being used.
Advanced Applications of Optical Amplifiers
- Long-Haul Communication: In long-haul fiber optic networks, Line Amplifiers (LAs) play a critical role. They are strategically placed at intervals to compensate for signal loss. For example, an LA with a high saturation output power of around +17 dBm and a low noise figure, typically less than 5 dB, can significantly extend the reach of the communication link without the need for electronic regeneration.
- Submarine Cables: Submarine communication cables, spanning thousands of kilometers, heavily rely on Distributed Amplifiers, like Raman amplifiers. These amplifiers uniquely boost the signal directly within the fiber, offering a more distributed amplification approach, which is crucial for such extensive undersea networks.
- Metropolitan Area Networks: In shorter, more congested networks like those in metropolitan areas, a combination of Booster Amplifiers (BAs) and Pre-amplifiers can be used. A BA, with an output power range of up to +23 dBm, can effectively launch a strong signal into the network, while a Pre-amplifier at the receiving end, with a very low noise figure (as low as 4 dB), enhances the receiver’s sensitivity to weak signals.
- Optical Add-Drop Multiplexers (OADMs): In systems using OADMs for channel multiplexing and demultiplexing, Line Amplifiers help in maintaining signal strength across the channels. The ability to handle multiple channels, each potentially with different power levels, is crucial. Here, the channel addition/removal (steady-state) gain response and transient gain response become significant parameters.
Technological Innovations and Challenges
The development of OA technologies is not without challenges. One of the primary concerns is managing the noise, especially in systems with multiple amplifiers. Each amplification stage adds some noise, quantified by the signal-spontaneous noise figure, which can accumulate and degrade the overall signal quality.
Another challenge is the management of Polarization Mode Dispersion (PMD) in Line Amplifiers. PMD can cause different light polarizations to travel at slightly different speeds, leading to signal distortion. Modern LAs are designed to minimize PMD, a critical parameter in high-speed networks.
Future of Optical Amplifiers in Industry
The future of OAs is closely tied to the advancements in fiber optic technology. As data demands continue to skyrocket, the need for more efficient, higher-capacity networks grows. Optical Amplifiers will continue to evolve, with research focusing on higher power outputs, broader wavelength ranges, and more sophisticated noise management techniques.
Innovations like hybrid amplification techniques, combining the benefits of Raman and Erbium-Doped Fiber Amplifiers (EDFAs), are on the horizon. These hybrid systems aim to provide higher performance, especially in terms of power efficiency and noise reduction.
References
ITU-T :https://www.itu.int/en/ITU-T/Pages/default.aspx
Image :https://www.chinacablesbuy.com/guide-to-optical-amplifier.html
Forward Error Correction (FEC) has become an indispensable tool in modern optical communication, enhancing signal integrity and extending transmission distances. ITU-T recommendations, such as G.693, G.959.1, and G.698.1, define application codes for optical interfaces that incorporate FEC as specified in ITU-T G.709. In this blog, we discuss the significance of Bit Error Ratio (BER) in FEC-enabled applications and how it influences optical transmitter and receiver performance.
The Basics of FEC in Optical Communications
FEC is a method of error control for data transmission, where the sender adds redundant data to its messages. This allows the receiver to detect and correct errors without the need for retransmission. In the context of optical networks, FEC is particularly valuable because it can significantly lower the BER after decoding, thus ensuring the accuracy and reliability of data across vast distances.
BER Requirements in FEC-Enabled Applications
For certain optical transport unit rates (OTUk), the system BER is mandated to meet specific standards only after FEC correction has been applied. The optical parameters, in these scenarios, are designed to achieve a BER no worse than 10â12 at the FEC decoder’s output. This benchmark ensures that the data, once processed by the FEC decoder, maintains an extremely high level of accuracy, which is crucial for high-performance networks.
Practical Implications for Network Hardware
When it comes to testing and verifying the performance of optical hardware components intended for FEC-enabled applications, achieving a BER of 10â12 at the decoder’s output is often sufficient. Attempting to test components at 10â12 at the receiver output, prior to FEC decoding, can lead to unnecessarily stringent criteria that may not reflect the operational requirements of the application.
Adopting Appropriate BER Values for Testing
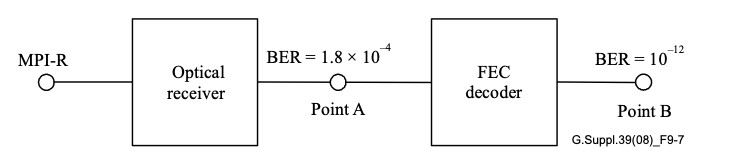
The selection of an appropriate BER for testing components depends on the specific application. Theoretical calculations suggest a BER of 1.8Ă10â4at the receiver output (Point A) to achieve a BER of 10â12 at the FEC decoder output (Point B). However, due to variations in error statistics, the average BER at Point A may need to be lower than the theoretical value to ensure the desired BER at Point B. In practice, a BER range of 10â5 to 10â6 is considered suitable for most applications.
Conservative Estimation for Receiver Sensitivity
By using a BER of 10â6 for component verification, the measurements of receiver sensitivity and optical path penalty at Point A will be conservative estimates of the values after FEC correction. This approach provides a practical and cost-effective method for ensuring component performance aligns with the rigorous demands of FEC-enabled systems.
Conclusion
FEC is a powerful mechanism that significantly improves the error tolerance of optical communication systems. By understanding and implementing appropriate BER testing methodologies, network operators can ensure their components are up to the task, ultimately leading to more reliable and efficient networks.
As the demands for data grow, the reliance on sophisticated FEC techniques will only increase, cementing BER as a fundamental metric in the design and evaluation of optical communication systems.
References
Signal integrity is the cornerstone of effective fiber optic communication. In this sphere, two metrics stand paramount: Bit Error Ratio (BER) and Q factor. These indicators help engineers assess the performance of optical networks and ensure the fidelity of data transmission. But what do these terms mean, and how are they calculated?
What is BER?
BER represents the fraction of bits that have errors relative to the total number of bits sent in a transmission. Itâs a direct indicator of the health of a communication link. The lower the BER, the more accurate and reliable the system.
ITU-T Standards Define BER Objectives
The ITU-T has set forth recommendations such as G.691, G.692, and G.959.1, which outline design objectives for optical systems, aiming for a BER no worse than 10â12 at the end of a system’s life. This is a rigorous standard that guarantees high reliability, crucial for SDH and OTN applications.
Measuring BER
Measuring BER, especially as low as 10â12, can be daunting due to the sheer volume of bits required to be tested. For instance, to confirm with 95% confidence that a system meets a BER of 10â12, one would need to test 3Ă1012 bits without encountering an error â a process that could take a prohibitively long time at lower transmission rates.
The Q Factor
The Q factor measures the signal-to-noise ratio at the decision point in a receiver’s circuitry. A higher Q factor translates to better signal quality. For a BER of 10â12, a Q factor of approximately 7.03 is needed. The relationship between Q factor and BER, when the threshold is optimally set, is given by the following equations:
The general formula relating Q to BER is:
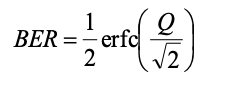
A common approximation for high Q values is:
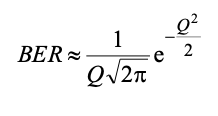
For a more accurate calculation across the entire range of Q, the formula is:
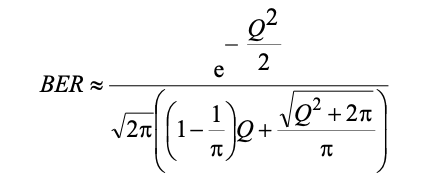
Practical Example: Calculating BER from Q Factor
Letâs consider a practical example. If a system’s Q factor is measured at 7, what would be the approximate BER?
Using the approximation formula, we plug in the Q factor:
This would give us an approximate BER thatâs indicative of a highly reliable system. For exact calculations, one would integrate the Gaussian error function as described in the more detailed equations.
Graphical Representation
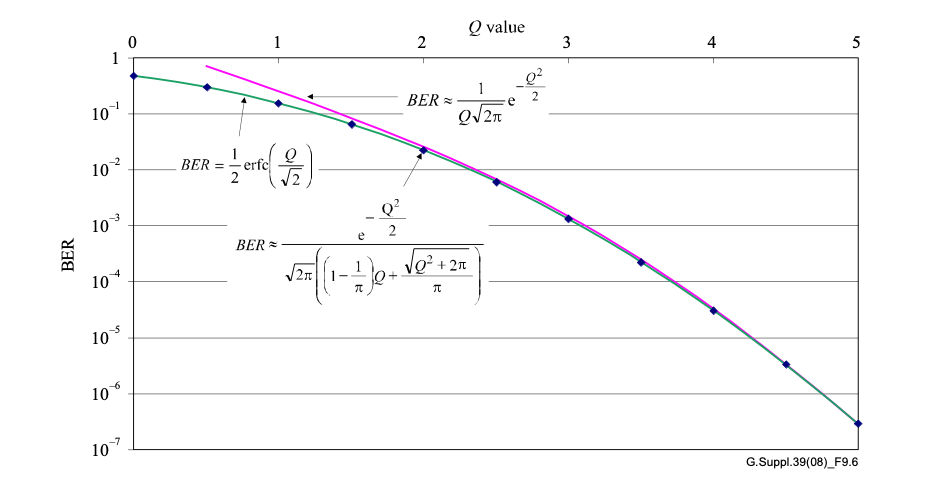
The graph typically illustrates these relationships, providing a visual representation of how the BER changes as the Q factor increases. This allows engineers to quickly assess the signal quality without long, drawn-out error measurements.
Concluding Thoughts
Understanding and applying BER and Q factor calculations is crucial for designing and maintaining robust optical communication systems. These concepts are not just academic; they directly impact the efficiency and reliability of the networks that underpin our modern digital world.
References
While single-mode fibers have been the mainstay for long-haul telecommunications, multimode fibers hold their own, especially in applications where short distance and high bandwidth are critical. Unlike their single-mode counterparts, multimode fibers are not restricted by cut-off wavelength considerations, offering unique advantages.
The Nature of Multimode Fibers
Multimode fibers, characterized by a larger core diameter compared to single-mode fibers, allow multiple light modes to propagate simultaneously. This results in modal dispersion, which can limit the distance over which the fiber can operate without significant signal degradation. However, multimode fibers exhibit greater tolerance to bending effects and typically showcase higher attenuation coefficients.
Wavelength Windows for Multimode Applications
Multimode fibers shine in certain “windows,” or wavelength ranges, which are optimized for specific applications and classifications. These windows are where the fiber performs best in terms of attenuation and bandwidth.
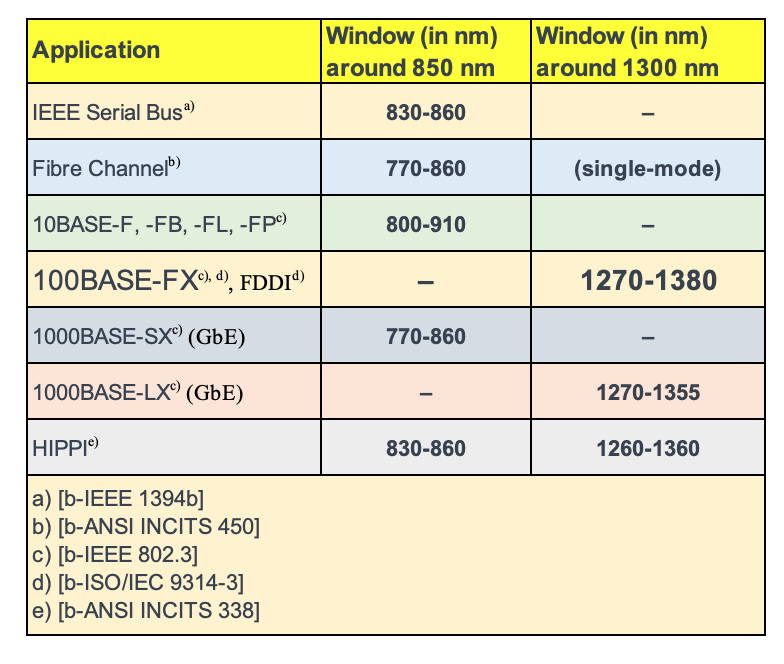
IEEE Serial Bus (around 850 nm): Typically used in consumer electronics, the 830-860 nm window is optimal for IEEE 1394 (FireWire) connections, offering high-speed data transfer over relatively short distances.
Fiber Channel (around 770-860 nm): For high-speed data transfer networks, such as those used in storage area networks (SANs), the 770-860 nm window is often used, although it’s worth noting that some applications may use single-mode fibers.
Ethernet Variants:
- 10BASE (800-910 nm): These standards define Ethernet implementations for local area networks, with 10BASE-F, -FB, -FL, and -FP operating within the 800-910 nm range.
- 100BASE-FX (1270-1380 nm) and FDDI (Fiber Distributed Data Interface): Designed for local area networks, they utilize a wavelength window around 1300 nm, where multimode fibers offer reliable performance for data transmission.
- 1000BASE-SX (770-860 nm) for Gigabit Ethernet (GbE): Optimized for high-speed Ethernet over multimode fiber, this application takes advantage of the lower window around 850 nm.
- 1000BASE-LX (1270-1355 nm) for GbE: This standard extends the use of multimode fibers into the 1300 nm window for Gigabit Ethernet applications.
HIPPI (High-Performance Parallel Interface): This high-speed computer bus architecture utilizes both the 850 nm and the 1300 nm windows, spanning from 830-860 nm and 1260-1360 nm, respectively, to support fast data transfers over multimode fibers.
Future Classifications and Studies
The classification of multimode fibers is a subject of ongoing research. Proposals suggest the use of the region from 770 nm to 910 nm, which could open up new avenues for multimode fiber applications. As technology progresses, these classifications will continue to evolve, reflecting the dynamic nature of fiber optic communications.
Wrapping Up: The Place of Multimode Fibers in Networking
Multimode fibers are a vital part of the networking world, particularly in scenarios that require high data rates over shorter distances. Their resilience to bending and capacity for high bandwidth make them an attractive choice for a variety of applications, from high-speed data transfer in industrial settings to backbone cabling in data centers.
As we continue to study and refine the classifications of multimode fibers, their role in the future of networking is guaranteed to expand, bringing new possibilities to the realm of optical communications.
References
Discover the most effective OSNR improvement techniques to boost the quality and reliability of optical communication systems. Learn the basics, benefits, and practical applications of OSNR improvement techniques today!
Introduction:
Optical signal-to-noise ratio (OSNR) is a key performance parameter that measures the quality of an optical communication system. It is a critical factor that determines the capacity, reliability, and stability of optical networks. To ensure optimal OSNR performance, various OSNR improvement techniques have been developed and implemented in modern optical communication systems.
In this article, we will delve deeper into the world of OSNR improvement techniques and explore the most effective ways to boost OSNR and enhance the quality of optical communication systems. From basic concepts to practical applications, we will cover everything you need to know about OSNR improvement techniques and how they can benefit your business.
So, let’s get started!
OSNR Improvement Techniques: Basics and Benefits
What is OSNR, and Why Does it Matter?
OSNR is a measure of the signal quality of an optical communication system, which compares the power of the signal to the power of the noise in the system. In simple terms, it is a ratio of the signal power to the noise power. A higher OSNR indicates a better signal quality and a lower error rate, while a lower OSNR indicates a weaker signal and a higher error rate.
OSNR is a critical factor that determines the performance and reliability of optical communication systems. It affects the capacity, reach, and stability of the system, as well as the cost and complexity of the equipment. Therefore, maintaining optimal OSNR is essential for ensuring high-quality and efficient optical communication.
What are OSNR Improvement Techniques?
OSNR improvement techniques are a set of methods and technologies used to enhance the OSNR performance of optical communication systems. They aim to reduce the noise level in the system and increase the signal-to-noise ratio, thereby improving the quality and reliability of the system.
There are various OSNR improvement techniques available today, ranging from simple adjustments to advanced technologies. Some of the most common techniques include:
- Optical Amplification: This technique involves amplifying the optical signal to increase its power and improve its quality. It can be done using various types of amplifiers, such as erbium-doped fiber amplifiers (EDFAs), Raman amplifiers, and semiconductor optical amplifiers (SOAs).
- Dispersion Management: This technique involves managing the dispersion properties of the optical fiber to minimize the pulse spreading and reduce the noise in the system. It can be done using various dispersion compensation techniques, such as dispersion-compensating fibers (DCFs), dispersion-shifted fibers (DSFs), and chirped fiber Bragg gratings (CFBGs).
- Polarization Management: This technique involves managing the polarization properties of the optical signal to minimize the polarization-mode dispersion (PMD) and reduce the noise in the system. It can be done using various polarization-management techniques, such as polarization-maintaining fibers (PMFs), polarization controllers, and polarization splitters.
- Wavelength Management: This technique involves managing the wavelength properties of the optical signal to minimize the impact of wavelength-dependent losses and reduce the noise in the system. It can be done using various wavelength-management techniques, such as wavelength-division multiplexing (WDM), coarse wavelength-division multiplexing (CWDM), and dense wavelength-division multiplexing (DWDM).
What are the Benefits of OSNR Improvement Techniques?
OSNR improvement techniques offer numerous benefits for optical communication systems, including:
- Improved Signal Quality: OSNR improvement techniques can significantly improve the signal quality ofthe system, leading to a higher data transmission rate and a lower error rate.
- Increased System Reach: OSNR improvement techniques can extend the reach of the system by reducing the impact of noise and distortion on the signal.
- Enhanced System Stability: OSNR improvement techniques can improve the stability and reliability of the system by reducing the impact of environmental factors and system fluctuations on the signal.
- Reduced Cost and Complexity: OSNR improvement techniques can reduce the cost and complexity of the system by allowing the use of lower-power components and simpler architectures.
Implementing OSNR Improvement Techniques: Best Practices
Assessing OSNR Performance
Before implementing OSNR improvement techniques, it is essential to assess the current OSNR performance of the system. This can be done using various OSNR measurement techniques, such as the optical spectrum analyzer (OSA), the optical time-domain reflectometer (OTDR), and the bit-error-rate tester (BERT).
By analyzing the OSNR performance of the system, you can identify the areas that require improvement and determine the most appropriate OSNR improvement techniques to use.
Selecting OSNR Improvement Techniques
When selecting OSNR improvement techniques, it is essential to consider the specific requirements and limitations of the system. Some factors to consider include:
- System Type and Configuration: The OSNR improvement techniques used may vary depending on the type and configuration of the system, such as the transmission distance, data rate, and modulation format.
- Budget and Resources: The cost and availability of the OSNR improvement techniques may also affect the selection process.
- Compatibility and Interoperability: The OSNR improvement techniques used must be compatible with the existing system components and interoperable with other systems.
- Performance Requirements: The OSNR improvement techniques used must meet the performance requirements of the system, such as the minimum OSNR level and the maximum error rate.
Implementing OSNR Improvement Techniques
Once you have selected the most appropriate OSNR improvement techniques, it is time to implement them into the system. This may involve various steps, such as:
- Upgrading or Replacing Equipment: This may involve replacing or upgrading components such as amplifiers, filters, and fibers to improve the OSNR performance of the system.
- Optimizing System Settings: This may involve adjusting the system settings, such as the gain, the dispersion compensation, and the polarization control, to optimize the OSNR performance of the system.
- Testing and Validation: This may involve testing and validating the OSNR performance of the system after implementing the OSNR improvement techniques to ensure that the desired improvements have been achieved.
FAQs About OSNR Improvement Techniques
What is the minimum OSNR level required for optical communication systems?
The minimum OSNR level required for optical communication systems may vary depending on the specific requirements of the system, such as the data rate, the transmission distance, and the modulation format. Generally, a minimum OSNR level of 20 dB is considered acceptable for most systems.
How can OSNR improvement techniques affect the cost of optical communication systems?
OSNR improvement techniques can affect the cost of optical communication systems by allowing the use of lower-power components and simpler architectures, thereby reducing the overall cost and complexity of the system.
What are the most effective OSNR improvement techniques for long-distance optical communication?
The most effective OSNR improvement techniques for long-distance optical communication may vary depending on the specific requirements and limitations of the system. Generally, dispersion compensation techniques, such as dispersion-compensating fibers (DCFs), and amplification techniques, such as erbium-doped fiber amplifiers (EDFAs), are effective for improving OSNR in long
distance optical communication.
Can OSNR improvement techniques be used in conjunction with other signal quality enhancement techniques?
Yes, OSNR improvement techniques can be used in conjunction with other signal quality enhancement techniques, such as forward error correction (FEC), modulation schemes, and equalization techniques, to further improve the overall signal quality and reliability of the system.
Conclusion
OSNR improvement techniques are essential for ensuring high-quality and reliable optical communication systems. By understanding the basics, benefits, and best practices of OSNR improvement techniques, you can optimize the performance and efficiency of your system and stay ahead of the competition.
Remember to assess the current OSNR performance of your system, select the most appropriate OSNR improvement techniques based on your specific requirements, and implement them into the system carefully and systematically. With the right OSNR improvement techniques, you can unlock the full potential of your optical communication system and achieve greater success in your business.
So, what are you waiting for? Start exploring the world of OSNR improvement techniques today and experience the power of high-quality optical communication!
Items | HD-FEC | SD-FEC |
---|---|---|
Definition | Decoding based on hard-bits(the output is quantized only to two levels) is called the âHD(hard-decision) decodingâ, where each bit is considered definitely one or zero. | Decoding based on soft-bits(the output is quantized to more than two levels) is called the âSD(soft-decision) decodingâ, where not only one or zero decision but also confidence information for the decision are provided. |
Application | Generally for non-coherent detection optical systems, e.g., Â 10 Gbit/s, 40 Gbit/s, also for some coherent detection optical systems with higher OSNR | coherent detection optical systems, e.g., Â 100 Gbit/s,400 Gbit/s. |
Electronics Requirement | ADC(Analogue-to-Digital Converter) is not necessary in the receiver. | ADC is required in the receiver to provide soft information, e.g., Â coherent detection optical systems. |
specification | general FEC per [ITU-T G.975];super FEC per [ITU-T G.975.1]. | vendor specific |
typical scheme | Concatenated RS/BCH | LDPC(Low density parity check),TPC(Turbo product code) |
complexity | medium | high |
redundancy ratio | generally 7% | around 20% |
NCG | about 5.6 dB for general FEC;>8.0 dB for super FEC. | >10.0 dB |
 Example(If you asked your friend about traffic jam status on roads and he replies) | maybe fully jammed or free |  50-50  but I found othe way free or less traffic |
Non-linear interactions between the signal and the silica fibre transmission medium begin to appear as optical signal powers are increased to achieve longer span lengths at high bit rates. Consequently, non-linear fibre behaviour has emerged as an important consideration both in high capacity systems and in long unregenerated routes. These non-linearities can be generally categorized as either scattering effects (stimulated Brillouin scattering and stimulated Raman scattering) or effects related to the fibre’s intensity dependent index of refraction (self-phase modulation, cross-phase modulation, modulation instability, soliton formation and four-wave mixing). A variety of parameters influence the severity of these non-linear effects, including line code (modulation format), transmission rate, fibre dispersion characteristics, the effective area and non-linear refractive index of the fibre, the number and spacing of channels in multiple channel systems, overall unregenerated system length, as well as signal intensity and source line-width. Since the implementation of transmission systems with higher bit rates than 10 Gbit/s and alternative line codes (modulation formats) than NRZ-ASK or RZ-ASK, described in [b-ITU-T G-Sup.39], nonâlinear fibre effects previously not considered can have a significant influence, e.g., intraâchannel cross-phase modulation (IXPM), intra-channel four-wave mixing (IFWM) and nonâlinear phase noise (NPN).
Optical power tolerance: It refers to the tolerable limit of input optical power, which is the range from sensitivity to overload point.
Optical power requirement: If refers to the requirement on input optical power, realized by adjusting the system (such as adjustable attenuator, fix attenuator, optical amplifier).
Optical power margin: It refers to an acceptable extra range of optical power. For example, ââ5/ + 3 dBâ requirement is actually a margin requirement.
General Causes of Bit Errors
- Â Performance degrade of key boards
- Abnormal optical power
- Signal-to-noise ratio decrease
- Non-linear factor
- Dispersion (chromatic dispersion/PMD) factor
- Optical reflection
- External factors (fiber, fiber jumper, power supply, environment and others)
The main advantages and drawbacks of EDFAs are as follows.
Advantages
- Commercially available in C band (1,530 to 1,565 nm) and L band (1,560 to 1,605) and up to  84-nm range at the laboratory stage.
- Excellent coupling: The amplifier medium is an SM fiber;
- Insensitivity to light polarization state;
- Low sensitivity to temperature;
- High gain: > 30 dB with gain flatness < Âą0.8 dB and < Âą0.5 dB in C and L band, respectively, in the scientific literature and in the manufacturer documentation
- Low noise figure: 4.5 to 6 dB
- No distortion at high bit rates;
- Simultaneous amplification of wavelength division multiplexed signals;
- Immunity to crosstalk among wavelength multiplexed channels (to a large extent)
Drawbacks
- Pump laser necessary;
- Difficult to integrate with other components;
- Need to use a gain equalizer for multistage amplification;
- Dropping channels can give rise to errors in surviving channels:dynamic control of amplifiers is  necessary.
The ITU standards define a âsuspect internal flagâ which should indicate if the data contained within a register is âsuspectâ (conditions defined in Q.822). This is more frequently referred to as the IDF (Invalid Data Flag).
PM is bounded by strict data collection rules as defined in standards. When the collection of PM parameters is affected then PM system labels the collection of data as suspect with an Invalid Data Flag (IDF). For the sake of identification; some unique flag is shown next to corresponding counter.
The purpose of the flag is to indicate when the data in the PM bin may not be complete or may have been affected such that the data is not completely reliable. The IDF does not mean the software is contingent.
Some of the common reasons for setting the IDF include:
- a collection time period that does not start within +/- 1 second of the nominal collection window start time.
- a time interval that is inaccurate by +/- 10 seconds (or more)
- the current time period changes by +/- 10 seconds (or more)
- a restart (System Controller restarts will wipe out all history data and cause time fluctuations at line/client module; Â a module restart will wipe out the current counts)
- a PM bin is cleared manually
- a hardware failure prevents PM from properly collecting a full period of PM data (PM clock failure)
- a protection switch has caused a change of payload on a protection channel.
- a payload reconfiguration has occurred (similar to above but not restricted to protection switches).
- an System Controller archive failure has occurred, preventing history data from being collected from the line/client  cards
- protection mode is switched from non-revertive to revertive (affects PSD only)
- a protection switch clear indication is received when no raise was indicated
- laser device failure (affects physical PMs)
- loss of signal (affects receive – OPRx, IQ – physical PMs only)
- Control Plane is booted less than 15 min period for 15-min interval and less than 24 hour period for 24-hour interval.
Suspect interval is determined by comparing nSamples to nTotalSamples on a counter PM. If nSamples is not equal to nTotalSamples then this period can be marked as suspect.Â
If any 15 minute is marked as suspect or reporting for that day interval is not started at midnight then it should flag that 24 Hr as suspect.
Some of the common examples are:
- Interface type is changed to another compatible interface (10G SR interface replaced by 10G DWDM interface),
- Line type is changed from SONET to SDH,
- Equipment failures are detected and those failures inhibit the accumulation of PM.
- Transitions to/from the ‘locked’ state.
- The System shall mark a given accumulation period invalid when the facility object is created or deleted during the interval.
- Node time is changed.
A short discussion on 980nm and 1480nm pump based EDFA
Introduction
The 980nm pump needs three energy level for radiation while 1480nm pumps can excite the ions directly to the metastable level .
(a) Energy level scheme of ground and first two excited states of Er ions in a silica matrix. The sublevel splitting and the lengths of arrows representing absorption and emission transitions are not drawn to scale. In the case of the 4 I11/2 state, s is the lifetime for nonradiative decay to the I13/2 first excited state and ssp is the spontaneous lifetime of the 4 I13/2 first excited state. (b) Absorption coefficient, a, and emission coefficient, g*, spectra for a typical aluminum co-doped EDF.
The most important feature of the level scheme is that the transition energy between the I15/2 ground state and the I13/2 first excited state corresponds to photon wavelengths (approximately 1530 to 1560 nm) for which the attenuation in silica fibers is lowest. Amplification is achieved by creating an inversion by pumping atoms into the first excited state, typically using either 980 nm or 1480 nm diode lasers. Because of the superior noise figure they provide and their superior wall plug efficiency, most EDFAs are built using 980 nm pump diodes. 1480 nm pump diodes are still often used in L-band EDFAs although here, too, 980 nm pumps are becoming more widely used.
Though pumping with 1480 nm is used and has an optical power conversion efficiency which is higher than that for 980 nm pumping, the latter is preferred because of the following advantages it has over 1480 nm pumping.
- It provides a wider separation between the laser wavelength and pump wavelength.
- 980 nm pumping gives less noise than 1480nm.
- Unlike 1480 nm pumping, 980 nm pumping cannot stimulate back transition to the ground state.
- 980 nm pumping also gives a higher signal gain, the maximum gain coefficient being 11 dB/mW against 6.3 dB/mW for the 1.48
- The reason for better performance of 980 nm pumping over the 1.48Â m pumping is related to the fact that the former has a narrower absorption spectrum.
- The inversion factor almost becomes 1 in case of 980 nm pumping whereas for 1480 nm pumping the best one gets is about 1.6.
- Quantum mechanics puts a lower limit of 3 dB to the optical noise figure at high optical gain. 980 nm pimping provides a value of 3.1 dB, close to the quantum limit whereas 1.48Â pumping gives a value of 4.2 dB.
- 1480nm pump needs more electrical power compare to 980nm.
Application
The 980 nm pumps EDFAâs are widely used in terrestrial systems while 1480nm pumps are used as Remote Optically Pumped Amplifiers (ROPA) in subsea links where it is difficult to put amplifiers.For submarine systems, remote pumping can be used in order not to have to electrically feed the amplifiers and remove electronic parts.Nowadays ,this is used in pumping up to 200km.
The erbium-doped fiber can be activated by a pump wavelength of 980 or 1480 nm but only the second one is used in repeaterless systems due to the lower fiber loss at 1.48 mm with respect to the loss at 0.98 mm. This allows the distance between the terminal and the remote amplifier to be increased.
In a typical configuration, the ROPA is comprised of a simple short length of erbium doped fiber in the transmission line placed a few tens of kilometers before a shore terminal or a conventional in-line EDFA. The remote EDF is backward pumped by a 1480 nm laser, from the terminal or in-line EDFA, thus providing signal gain
Vendors
Following are the vendors that manufactures 980nm and 1480nm EDFAs
As we know that either homodyne or heterodyne detection can be used to convert the received optical signal into an electrical form. In the case of homodyne detection, the optical signal is demodulated directly to the baseband. Although simple in concept, homodyne detection is difficult to implement in practice, as it requires a local oscillator whose frequency matches the carrier frequency exactly and whose  phase is locked to the incoming signal. Such a demodulation scheme is called synchronous and is essential for homodyne detection. Although optical phase-locked loops have been developed for this purpose, their use is complicated in practice.
Heterodyne detection simplifies the receiver design, as neither optical phase locking nor frequency matching of the local oscillator is required. However, the electrical signal oscillates rapidly at microwave frequencies and must be demodulated from the IF bandto the baseband using techniques similar to those developed for microwave communication systems. Demodulation can be carried out either synchronously or asynchronously. Asynchronous demodulation is also called incoherent in the radio communication literature. In the optical communication literature, the term coherent detection is used in a wider sense.
A lightwave system is called coherent as long as it uses a local oscillator irrespective of the demodulation technique used to convert the IF signal to baseband frequencies.
*In case of homodyne coherent-detection technique, the local-oscillator frequency is selected to coincide with the signal-carrier frequency.
*In case of heterodyne detection the local-oscillator frequency  is chosen to differ from the signal-carrier frequency.
What Is Coherent Communication?
Definition of coherent light
A coherent light consists of two light waves that:
1) Have the same oscillation direction.
2) Have the same oscillation frequency.
3) Have the same phase or maintain a constant phase relationship with each other. Two coherent light waves produce interference within the area where they meet.
Principles of Coherent Communication
Coherent communication technologies mainly include coherent modulation and coherent detection.
Coherent modulation uses the signals that are propagated to change the frequencies, phases, and amplitudes of optical carriers. (Intensity modulation only changes the strength of light.)
Modulation detection mixes the laser light generated by a local oscillator (LO) with the incoming signal light using an optical hybrid to produce an IF signal that maintains the constant frequency, phase, and amplitude relationships with the signal light.
Â
Â
The motivation behind using the coherent communication techniques is two-fold.
First, the receiver sensitivity can be improved by up to 20 dB compared with that of IM/DD systems.
Second, the use of coherent detection may allow a more efficient use of fiber bandwidth by increasing the spectral efficiency of WDM systems
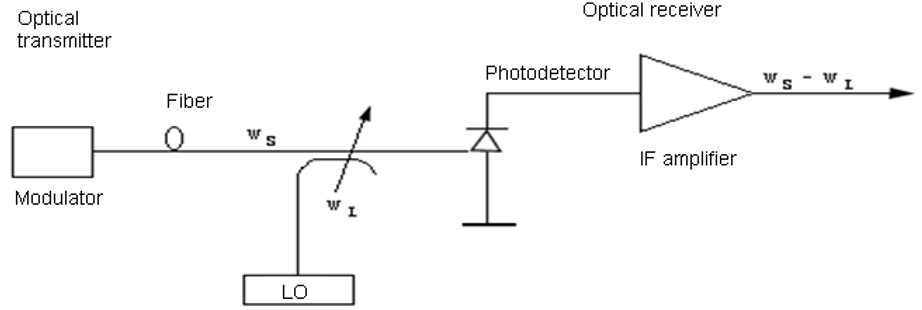
In a non-coherent WDM system, each optical channel on the line side uses only one binary channel to carry service information. The service transmission rate on each optical channel is called bit rate while the binary channel rate is called baud rate. In this sense, the baud rate was equal to the bit rate. The spectral width of an optical signal is determined by the baud rate. Specifically, the spectral width is linearly proportional to the baud rate, which means a higher baud rate generates a larger spectral width.
- Baud (pronounced as /bÉ:d/ and abbreviated as “Bd”) is the unit for representing the data communication speed. It indicates the signal changes occurring in every second on a device, for example, a modulator-demodulator (modem). During encoding, one baud (namely, the signal change) actually represents two or more bits. In the current high-speed modulation techniques, each change in a carrier can transmit multiple bits, which makes the baud rate different from the transmission speed.
In practice, the spectral width of the optical signal cannot be larger than the frequency spacing between WDM channels; otherwise, the optical spectrums of the neighboring WDM channels will overlap, causing interference among data streams on different WDM channels and thus generating bit errors and a system penalty.
For example, the spectral width of a 100G BPSK/DPSK signal is about 50 GHz, which means a common 40G BPSK/DPSK modulator is not suitable for a 50 GHz channel spaced 100G system because it will cause a high crosstalk penalty. When the baud rate reaches 100 Gbaud/s, the spectral width of the BPSK/DPSK signal is greater than 50 GHz. Thus, it is impossible to achieve 50 GHz channel spacing in a 100G BPSK/DPSK transmission system.
(This is one reason that BPSK cannot be used in a 100G coherent system. The other reason is that high-speed ADC devices are costly.)
A 100G coherent system must employ new technology. The system must employ more advanced multiplexing technologies so that an optical channel contains multiple binary channels. This reduces the baud rate while keeping the line bit rate unchanged, ensuring that the spectral width is less than 50 GHz even after the line rate is increased to 100 Gbit/s. These multiplexing technologies include quadrature phase shift keying (QPSK) modulation and polarization division multiplexing (PDM).
Perform the following operations to measure OSNR using the integral method:
In this example, OSNR = 10 x log[(9.68 – 0.02958)/0.007395] = 31.156 dB
We follow integral method because Direct OSNR Scanning Cannot Ensure Accuracy because of the following reason:
A 40G/100G signal has a larger spectral width than a 10G signal. As a result, the signal spectrums of adjacent channels overlap each other. This brings difficulties in testing the OSNR using the traditional OSA method, which is implemented based on the interpolation of inter-channel noise that is equivalent to in-band noise. Inter-channel noise power contains not only the ASE noise power but also the signal crosstalk power. Therefore, the OSNR obtained using the traditional OSA method is less than the actual OSNR. The figure below shows the signal spectrums in hybrid transmission of 40G and 10G signals with 50 GHz channel spacing. As shown in the figure, a severe spectrum overlap has occurred and the tested ASE power is greater than it should be .As ROADM and OEQ technologies become mature and are widely used, the use of filter devices will impair the noise spectrum. As shown in the following figure, the noise power between channels decreases remarkably after signals traverse a filter. As a result, the OSNR obtained using the traditional OSA method is greater than the actual OSNR..
Basic understanding on Tap ratio for Splitter/Coupler
Fiber splitters/couplers divide optical power from one common port to two or more split ports and combine all optical power from the split ports to one common port (1 Ă N coupler). They operate across the entire band or bands such as C, L, or O bands. The three port 1 Ă 2 tap is a splitter commonly used to access a small amount of signal power in a live fiber span for measurement or OSA analysis. Splitters are referred to by their splitting ratio, which is the power output of an individual split port divided by the total power output of all split ports. Popular splitting ratios are shown in Table below; however, others are available. Equation below can be used to estimate the splitter insertion loss for a typical split port. Excess splitter loss adds to the port’s power division loss and is lost signal power due to the splitter properties. It typically varies between 0.1 to 2 dB, refer to manufacturerâs specifications for accurate values. It should be noted that splitter function is symmetrical.
where IL = splitter insertion loss for the split port, dB
Pi = optical output power for single split port, mW
PTÂ = total optical power output for all split ports, mW
SR = splitting ratio for the split port, %
Îe = splitter excess loss (typical range 0.1 to 2 dB), dB
Common splitter applications include
⢠Permanent installation in a fiber link as a tap with 2%|98% splitting ratio. This provides for access to live fiber signal power and OSA spectrum measurement without affecting fiber traffic. Commonly installed in DWDM amplifier systems.
⢠Video and CATV networks to distribute signals.
⢠Passive optical networks (PON).
⢠Fiber protection systems.
Example with calculation:
If a 0 dBm signal is launched into the common port of a 25% |75% splitter, then the two split ports, output power will be â6.2 and â1.5 dBm. However, if a 0 dBm signal is launched into the 25% split port, then the common port output power will be â6.2 dBm.
Calculation.
Launch power=0 dBm =1mW
      Â
Tap is  25%|75%
so equivalent mW power which is linear  will be
0.250mW|0.750mW
and after converting them ,dBm value will be
-6.02dBm|Â -1.24dBm
Some of the common split ratios and their equivalent Optical Power is available below for reference.
Q is the quality of a communication signal and is related to BER. A lower BER gives a higher Q and thus a higher Q gives better performance. Q is primarily used for translating relatively large BER differences into manageable values.
Pre-FEC signal fail and Pre-FEC signal degrade thresholds are provisionable in units of dBQ so that the user does not need to worry about FEC scheme when determining what value to set the thresholds to as the software will automatically convert the dBQ values to FEC corrections per time interval based on FEC scheme and data rate.
The Q-Factor, is in fact a metric to identify the attenuation in the receiving signal and determine a potential LOS and it is an estimate of the Optical-Signal-to-Noise-Ratio (OSNR) at the optical receiver.  As attenuation in the receiving signal increases, the dBQ value drops and vice-versa. Hence a drop in the dBQ value can mean that there is an increase in the Pre FEC BER, and a possible LOS could occur if the problem is not corrected in time.
The Quality of an Optical Rx signal can be measured by determining the number of “bad” bits in a block of received data. The bad bits in each block of received data are removed and replaced with “good” zero’s or one’s such that the network path data can still be properly switched and passed on to its destination. This strategy is referred to as Forward Error Correction (FEC) and prevents a complete loss of traffic due to small un-important data-loss that can be re-sent again later on. The process by which the “bad” bits are replaced with the “good” bits in an Rx data block is known as Mapping. The Pre FEC are the FEC Counts of “bad” bits before the Mapper and the FEC Counts (or Post FEC Counts) are those after the Mapper.
The number of Pre FEC Counts for a given period of time can represent the status of the Optical Rx network signal; An increase in the Pre FEC count means that there is an increase in the number of “bad” bits that need to be replaced by the Mapper. Hence a change in rate of the Pre FEC Count (Bit Erro Rate – BER) can identify a potential problem upstream in the network. At some point the Pre FEC Count will be too high as there will be too many “bad” bits in the incoming data block for the Mapper to replace … this will then mean a Loss of Signal (LOS).
As the normal number of Pre FEC Counts are high (i.e. 1.35E-3 to 6.11E-16) and constantly fluctuate, it can be difficult for an network operator to determine whether there is a potential problem in the network. Hence a dBQ value, known as the Q-Factor, is used as a measure of the Quality of the receiving optical signal. It should be consistent with the Pre FEC Count Bit Error Rate (BER).
The standards define the Q-Factor as Q = 10log[(X1 – X0)/(N1 – N0)] where Xj and Nj are the mean and standard deviation of the received mark-bit (j=1) and space-bit (j=0) ……………. In some cases Q = 20log[(X1 – X0)/(N1 – N0)]
For example, the linear Q range 3 to 8 covers the BER range of 1.35E-3 to 6.11E-16.
Nortel defines dBQ as 10xlog10(Q/Qref) where Qref is the pre-FEC raw optical Q, which gives a BER of 1E-15 post-FEC assuming a particular error distribution. Some organizations define dBQ as 20xlog10(Q/Qref), so care must be taken when comparing dBQ values from different sources.
The dBQ figure represents the dBQ of margin from the following pre-FEC BERs (which are equivalent to a post-FEC BER of 1E-15). The equivalent linear Q value for these BERs are  Qref in the above formula.
Pre-FEC signal degrade can be used the same way a car has an âoil lightâ in that it states that there is still margin left but you are closer to the fail point than expected so action should be taken.
The Optical Time Domain Reflectometer (OTDR) is useful for testing the integrity of fiber optic cables. An optical time-domain reflectometer (OTDR) is an optoelectronic instrument used to characterize an optical fiber. An OTDR is the optical equivalent of an electronic time domain reflectometer. It injects a series of optical pulses into the fiber under test. It also extracts, from the same end of the fiber, light that is scattered (Rayleigh backscatter) or reflected back from points along the fiber. The strength of the return pulses is measured and integrated as a function of time, and plotted as a function of fiber length.
Using an OTDR, we can:
1. Measure the distance to a fusion splice, mechanical splice, connector, or significant bend in the fiber.
2. Measure the loss across a fusion splice, mechanical splice, connector, or significant bend in the fiber.
3. Measure the intrinsic loss due to mode-field diameter variations between two pieces of single-mode optical fiber connected by a splice or connector.
4. Determine the relative amount of offset and bending loss at a splice or connector joining two single-mode fibers.
5. Determine the physical offset at a splice or connector joining two pieces of single-mode fiber, when bending loss is insignificant.
6. Measure the optical return loss of discrete components, such as mechanical splices and connectors.
7. Measure the integrated return loss of a complete fiber-optic system.
8. Measure a fiberâs linearity, monitoring for such things as local mode-field pinch-off.
9. Measure the fiber slope, or fiber attenuation (typically expressed in dB/km).
10. Measure the link loss, or end-to-end loss of the fiber network.
11. Measure the relative numerical apertures of two fibers.
12. Make rudimentary measurements of a fiberâs chromatic dispersion.
13. Measure polarization mode dispersion.
14. Estimate the impact of reflections on transmitters and receivers in a fiber-optic system.
15. Provide active monitoring on live fiber-optic systems.
16. Compare previously installed waveforms to current traces.
The maintenance signals defined in [ITU-T G.709] provide network connection status information in the form of payload missing indication (PMI), backward error and defect indication (BEI, BDI), open connection indication (OCI), and link and tandem connection status information in the form of locked indication (LCK) and alarm indication signal (FDI, AIS).
Interaction diagrams are collected from ITU G.798 and OTN application note from IpLight